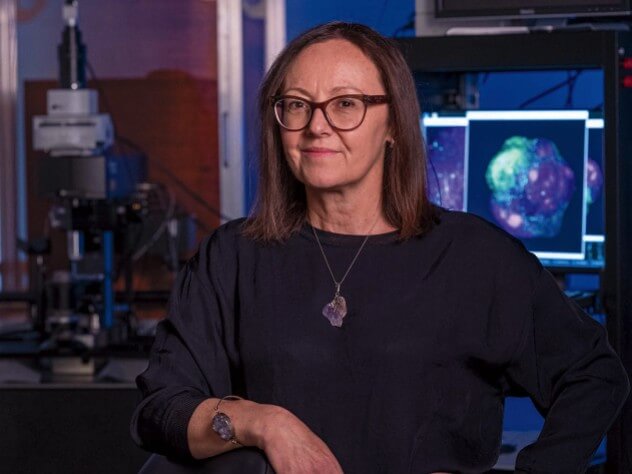
This article originally appeared in Harvard Magazine.
Paola Arlotta is searching for the words to describe the clusters of cells, incubating quietly in a small room next to her laboratory, that have profoundly reshaped her life’s work—not just the how or how much or how fast of her research, but the very concept of what science is possible.
“Like an apple seed,” she says finally, “or maybe a very, very small raisin. They’re tiny, but you can see them with your eye.”
She’s talking about brain organoids, three-dimensional tissues grown from human stem cells that mimic aspects of an actual brain. They’re still smaller and simpler than the real thing, but watching organoids grow in laboratory cultures—seeing neural cells develop together and assemble themselves into networks and circuits, much the way they do in life—has given scientists their first direct glimpse into the early formation of the human brain. “I cannot tell you how exciting this is,” says Arlotta, one of the field’s foremost researchers. “We have never been able to study this before, because it all happens in the womb.” But now, she says, “I can actually see the cells being formed, the human-specific cells that I cannot find in a mouse or even a macaque. It’s priceless.”
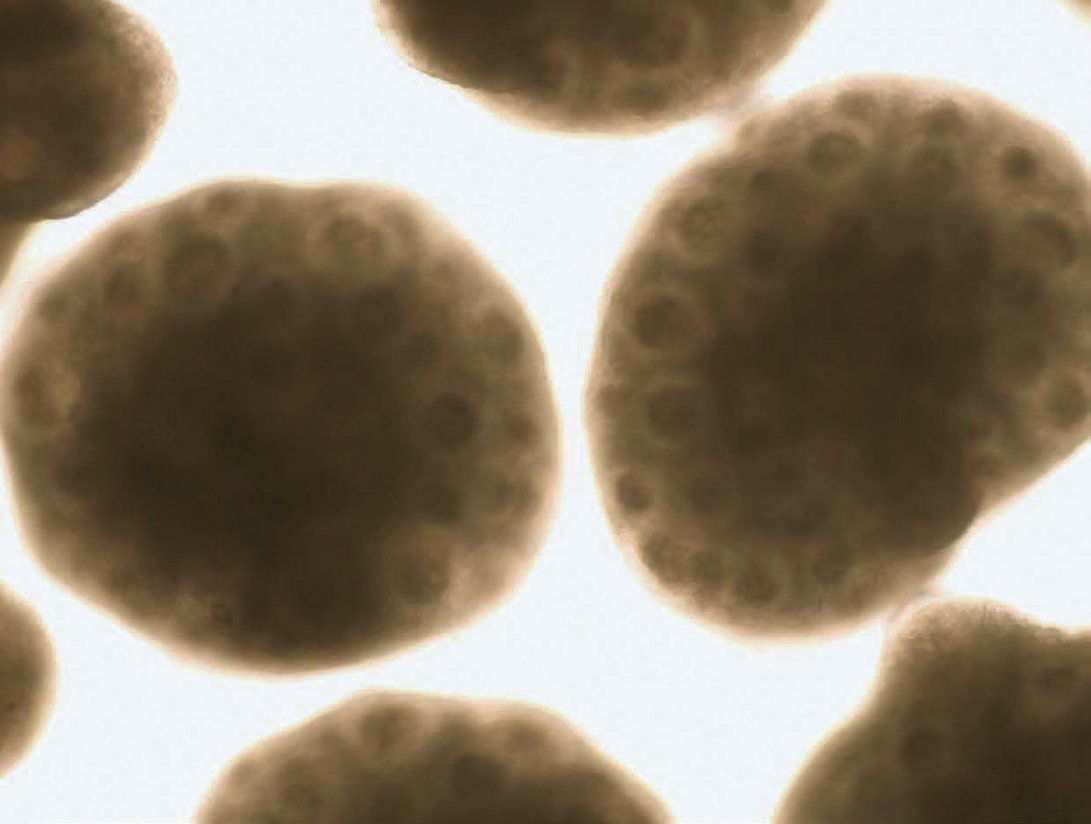
Dark-haired and dimpled, with a lilting Italian accent and a storyteller’s instinct for timing and precision, Arlotta radiates a buoyant, infectious intensity. The Golub Family professor and chair of the department of stem cell and regenerative biology, she is also a member of the Stanley Center for Psychiatric Research at the Broad Institute of MIT and Harvard. Her work with organoids ties together all these fields, and already it has begun to yield breakthroughs in the understanding of the human brain: its elegance and intricacy and inner workings, but also its troubles.
The organoids she builds model the cerebral cortex, responsible for higher functions including language, memory, reasoning, emotion, decision-making, and personality. It’s also the part of the brain affected by disorders like autism, schizophrenia, and dementia, and much of the work in Arlotta’s lab is geared toward figuring out what happens in those cases—and finding clues for how doctors might someday intervene. “What cell types are involved in the brain of an autistic kid?” she says. “What are the mechanisms? What are the convergences? Nobody knows.” But in her laboratory and others like it, researchers are starting to generate answers. The work, she says, is like cracking open a “black box.”
Organoids offer a startlingly powerful tool for these discoveries. When a child begins showing signs of autism, Arlotta says, it’s likely that the disorder began before birth, during the first weeks or months of gestation. “We can’t go back to that process and see what happened,” she says. “It’s gone.” But by taking a sample of the patient’s blood or skin, transforming it into pluripotent stem cells that can grow neural tissue, and then watching an organoid develop—in which every single cell carries the patient’s own genome—scientists can reenact a version of that original process from the womb. “It’s like replaying a movie,” she says. “And if you watch, you may catch the defect.”
Some scientists are also beginning to incorporate organoids into drug-development research. Neuroscience has always been a tricky area for pharmaceutical work: neurological diseases are complex and difficult to unravel, and our knowledge of the brain is limited. Mice, often used as a proxy, closely resemble human biology in many ways, but there are real differences—especially in the brain and spinal cord. “So, many of the drugs that can cure a mouse fail once they go into human clinical trials,” Arlotta notes. As organoids become more sophisticated and precise, they could be used to screen drugs ahead of trials: determining which ones are effective, but also teasing out how genetic differences affect patients’ responses and identifying the groups of patients most likely to benefit. “And you could actually screen the drugs on the very tissue of the patient that you’re trying to treat,” she says.
Up close, organoids look strikingly small and delicate, with a gossamer, almost ghostly, beauty. Arlotta offers a glimpse one afternoon in her laboratory. In latex gloves and lab coats, postdoc Rahel Kastli and technician Sophia Andreadis open a refrigerator-sized incubator and lift out a culture dish containing dozens of round, translucent, soft-looking beads suspended in a watery pink medium. Produced a little less than a month earlier, they’re extremely tiny, closer to the size of a mustard seed than an apple seed. Another incubator contains slightly larger specimens, perhaps four millimeters across, which Arlotta’s team have been growing for four years. They look like pale, miniature lentils floating at the bottom of a bioreactor flask that keeps them constantly in motion. (The oldest organoids in the lab—more fragile, and usually kept locked away—have been developing for seven years.)
Organoids, Arlotta explains, mature slowly, at roughly the same rate as real brains—so inexorably is the human time scale written into their DNA. Which is why studying them requires patience. “This is not science that is done overnight,” she says. “It takes a long time, at least six months, to make all of the cells of the cerebral cortex in culture. So, you have to commit to it for many, many years—potentially your career—because the investment in it is so long.”
But she also sees something beautiful in that process—something exhilarating and poignant in biology’s refusal to be rushed, its insistence, always, on its own clock.
GROWING UP in a village of 1,500 people in far northeastern Italy, Arlotta was hardly destined to become a scientist. “Most people there made wine—it’s a very good region for that,” she says. Her parents were middle-class government employees, and although aunts and uncles and cousins fanned out into various fields, she and her brother were the only ones who went to college. He studied business. Arlotta, who as a child spent hours watching worms in the garden, decided to try biology at the University of Trieste. She’d had a science teacher in high school, Professor Vecchia, whose lessons had lit a fire. “Instead of simply teaching,” she remembers, “he would build little experiments for us, sometimes silly ones, to surprise us in some way or to describe some concept. And then he would say, ‘What do you see?’” She still has the notes he handed out in class. “To be in a public school in the middle of nowhere with perhaps four kids in the class who were interested, he still did all this,” she says. “I loved it. I couldn’t leave the lab. I don’t know how many times I went back after school.”
At Trieste, she discovered an interest in embryology. “How does an embryo build…us?” she says, almost whispering a question whose answer still astonishes her decades later. After earning her bachelor’s degree, she stayed for a master’s in biochemistry, and then moved to the University of Portsmouth, in England, to pursue a Ph.D. in molecular biology. She first arrived at Harvard as a postdoc at Harvard Medical School, and it was there that she fell into the study of the brain. “It’s the organ that defines us,” she says. “It’s who we are. And it keeps evolving.”
Later, in her own lab on campus, Arlotta spent years studying the development of the cerebral cortex of mice, as a model for the human brain. Her findings sometimes upended long-held assumptions. A 2013 study demonstrated that neurons could be “reprogrammed” from one type to another within the living brains of young mice, opening the possibility that malfunctioning neural circuits could be rewired. In other research, she helped show that myelin, the nervous system’s insulating material—lost or damaged in diseases like multiple sclerosis and schizophrenia—is less widespread in the brain than previously thought, especially in complex regions of the higher cortex, which evolved most recently.
A few years ago, she and several colleagues published a detailed “atlas” of the mouse somatosensory cortex, which processes sensory information from the body, such as touch, pain, or heat. The researchers profiled all its cells and cataloged minute changes in gene expression and regulation during each day of embryonic development. The study provided the first dynamic look at what Arlotta often calls the “symphony” of brain formation, in which cell types are created in specific locations at specific times, interacting with each other in tightly orchestrated ways to form “this beautiful machine.”
At a certain point, though, the work began to feel like “a bit of a selfish exercise,” she says. It no longer seemed enough to keep investigating fundamental aspects of brain development, filling in the next chapter of scientific knowledge for its own sake. Arlotta began thinking about neurodevelopmental disorders, and how her past research might help understand and treat them. “These are very difficult diseases, right?” she says. “We know very little about them, and the need is very clear. You look at the lack of new therapies in the last 70 years for schizophrenia”—the most commonly used neuroleptic medications were first developed in the early 1950s—“and that’s a very real problem.” She felt particularly drawn to the conundrum of autism. “I’ve seen what that condition can be,” she says. “How difficult it is for the families. Morally, I just couldn’t stay still.”
Arlotta’s change of direction coincided with a breakthrough in organoids research in 2013, when the prospect of creating workable stem-cell-based models of the human brain became real. The tissues were rudimentary, but “They were powerful,” she says. “They opened up the field.” A revolution in single-cell genomics technologies opened the field up further, enabling scientists to take a tissue sample from anywhere in the body, break it down, and determine what genes were being produced in the individual cells. “And so now all of a sudden,” she recalls, “without knowing anything about the [organoid] tissue that I’m making—because at the beginning there was so much we didn’t know—I can characterize that tissue and say, ‘Oh, it makes this type of cell, it makes that type of cell, but it doesn’t make this other one. And how do we study that?’”
Much of Arlotta’s research since has been devoted to figuring out ways to make organoids more reliable and usable in experiments. In 2017, she coauthored a paper outlining protocols and culture conditions to keep them developing for nine months or more (back then, most lasted only weeks). She and her collaborators also found that the longer the organoids matured, the wider the diversity of neural cell types they generated, reflecting the way development works inside the body. The organoids in the study produced spontaneously active neuronal networks; dendritic spines, which help transmit electric signals to the neurons; and a nearly complete array of cell types from the retina, including photosensitive cells. When the researchers exposed the tissue to light, the cells reacted.
IN 2019 came a major advance: Arlotta and her team discovered a method for creating organoids that are virtually indistinguishable from one another and that consistently produce the same types of cells, in the same order, and along the same trajectory as real human cerebral cortexes. This was a dramatic shift. Until then, organoids had varied widely, each one “its own snowflake,” she says, generating its own combination of cell types in unpredictable ways, which made it difficult for scientists to use them as experimental models in studies of disease. Arlotta and her coauthors cultured organoids from a number of different stem cell lines, both male and female, with different genetic backgrounds; despite those varied origins, the tissues that emerged were consistent. “Truly, it was a huge step,” says Steven Hyman, director of the Stanley Center for Psychiatric Research and distinguished service professor of stem cell and regenerative biology. “If you want to learn what a gene does, it can’t be studied sensibly in a background that is full of noise….The ability to make organoids that overcome damaging technical variability is just absolutely critical to the field.”
Other critical advances still await. For the past few years, Arlotta has worked with materials scientist Jennifer Lewis and other Harvard researchers on an effort to build organoids with functioning networks of blood vessels. The tissues can survive for years, but without a blood supply, their ability to expand and mature is limited. “We want to make them more physiologically relevant, to understand how the vasculature influences organoid development,” says Lewis, the Wyss professor of biologically inspired engineering. A vascular system would also allow scientists to link organoids that mimic different brain regions—the hippocampus, the thalamus, the pituitary, the cerebellum—and see them interact. “The brain is not just a tiny piece of cortex,” Arlotta says. “It’s cortex connected to other parts of the brain that are receiving inputs from a sensory organ.”
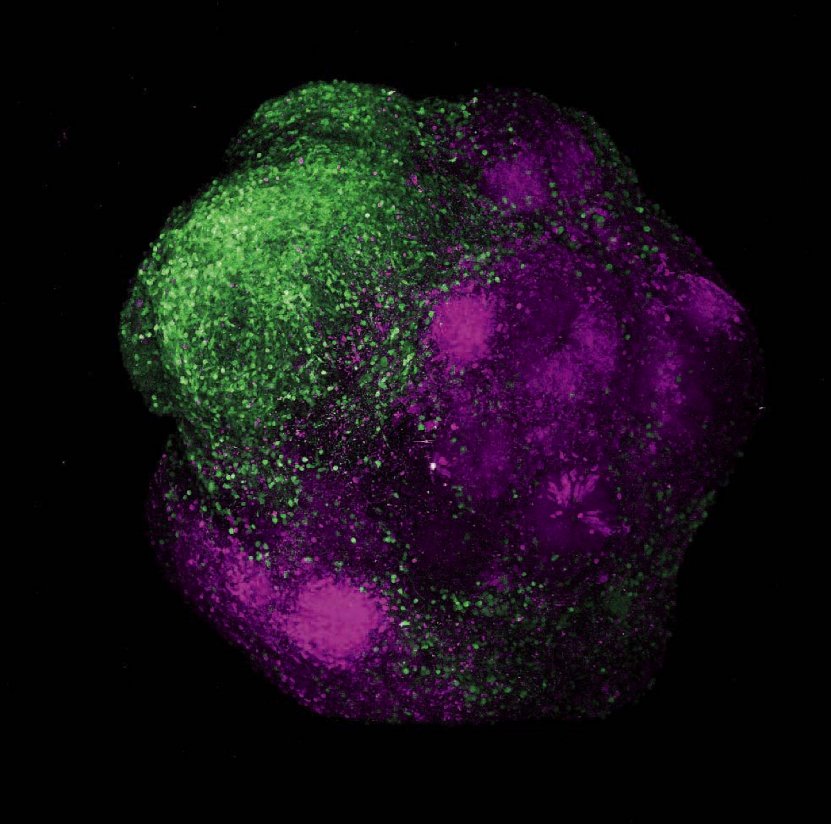
She has also begun studying neurological disorders more directly. In 2022, Arlotta published the results of a five-year study on autism, in which she and her team analyzed organoids that had been altered with three genetic mutations known to occur in autistic patients. One longstanding hypothesis about autism suggests that the disorder is based on an imbalance between excitatory and inhibitory mechanisms in the cerebral cortex, which regulate the firing of neural circuits. Arlotta found that in the mutated organoids, inhibitory neurons were developing too fast relative to their partner neurons. “And in this symphony of brain formation, when you begin to have cells whose timing is not coordinated,” Arlotta says, “what you risk is…that the circuit, the network they form with other cell types, may be abnormal.” And indeed, she discovered that organoids grown with autism mutations produced abnormal networks. This work is only a first step, she adds. “Now I can put a name to the cells affected. It gives some information to start thinking about therapeutic intervention—and the next set of studies—in an informed way.”
That includes her own next studies. Among the findings Arlotta’s team reported was that while organoids from different stem-cell donors were all affected in the same way by the autism mutations, the severity of those effects varied. “Genetic context matters,” she explains. Some genomes buffer a disease mutation, while others don’t. This realization gave her an idea: What if scientists could build a “mosaic” organoid incorporating genetic material from many individuals? So that by testing a single piece of tissue, “you might have a window into understanding how the cells of different people respond to drugs, therapeutics, genetics, environment, stressors—you name it—all at once,” she says. Such a mosaic might allow researchers to predict treatment outcomes, design better clinical trials, and untangle what enables one genome to counter a disease mutation, while another cannot. “I think this is extremely powerful,” she says. It’s one of the many projects her lab is working on.
FROM THE BEGINNING, scientists like Arlotta have been aware of an oncoming ethical dilemma. That’s because there is a Catch-22 at the heart of brain organoid research: in order to help understand and find cures for neurological disease, the tissues must resemble real human brains as closely as possible. But the closer they come to real brains, the greater the chance of crossing the kind of moral line that prevents scientists from experimenting on living humans. Some advances have sparked particular debate. Organoids can now produce nearly every kind of brain cell, and some, like the photosensitive tissues in Arlotta’s lab, exhibit behaviors in response to stimuli; elsewhere, scientists have implanted organoids into rats and mice. And as the effort progresses to create organoids with their own vascular systems, allowing them to mature more fully and mimic more complex neural functions, that breakthrough will also trigger complicated questions.
In both the popular imagination and scientific debate, some questions inevitably arise: could organoids someday become conscious or feel pain? Could they achieve self-awareness? Those are concerns to take seriously, Arlotta says, but for the moment, she is not worried. Near-unanimous consensus among those working in the field—which includes not only scientists but also bioethicists and philosophers—is that organoids are still a long way from consciousness. The more immediate concerns involve issues like the informed consent of stem-cell donors, the limits of appropriate use for organoids, and the ability to validate their effectiveness as brain models—each of which opens up its own web of fine-grained and fundamental moral questions.
“It’s important to understand the science for what it is,” Arlotta says. “And it’s important to communicate what the systems are and where they can go—or where we imagine they can go.” Organoids don’t yet capture the complexity of real brains; the most advanced versions mimic late embryonic or early postnatal tissue, she says, “not adult brains.” They lack the sensory experiences that help shape the circuitry and development of a brain attached to a body. And although organoids can grow most types of neural cells, those cells don’t yet organize into the structures normally seen in the brain.
But, Arlotta cautions, “It’s only a matter of time, right? Because the science moves fast.” These days, she works with bioethicists whenever she’s applying for a new research grant: “You don’t do these experiments without having a very clear ethical framework for your work.” In her vascularization project with Lewis, one of the principal collaborators is Insoo Hyun, a member of Harvard’s Center for Bioethics and director of life sciences at the Boston Museum of Science. He sees his role as a kind of continuous dissection: exposing unfounded assumptions or reasoning that jumps to conclusions, and resisting the impulse to over-theorize. Echoing Arlotta, he says it’s important for bioethicists to know “the underlying biology, the actual science.”
Recently, Arlotta was one of three dozen scientists, bioethicists, and legal scholars who spent a year studying these issues for the National Academies of Sciences, Engineering and Medicine; in 2021 the committee produced a book-length report outlining a list of ethical concerns and suggested approaches for regulation and governance, building on existing rules and frameworks that apply to other kinds of biological and stem-cell research. She also took part in a two-year program, the Brainstorm Project, which was led by Hyun and Jeantine Lunshof, a philosopher and ethicist at the Wyss Institute for Biologically Inspired Engineering and Harvard Medical School lecturer. Funded by the National Institutes of Health, the project gathered scientists and bioethicists into working groups to discuss issues and make recommendations about future research. It was an exercise in what Lunshof calls “collaborative ethics,” which she sees as the future of organoid research: scientists and ethicists in continuous conversation, developing the field together. “It’s important that we have this ethical conversation,” Arlotta says, “and that we keep having it. One of the things we emphasized is the need to constantly evaluate what we do.”
And yet. It’s also important—and ethically imperative, Arlotta believes—to keep going. “There is huge value in this work,” she says. “There is the possibility to alleviate a tremendous amount of suffering.” In the quest to understand neural disease, those tiny clusters of cells incubating in her lab are not “some sort of incremental advance,” she says. “This is a whole different thing.”